Environment and Energy
Microscopically Exploring the Basis of Human Survival
Making the Impossible Possible with Highly Brilliant, Highly Collimated, and Wavelength Tunable Synchrotron Radiation
In today's world almost everything consumes energy, and modern society is under immense pressure to actively reduce mankind's impact on the environment. Many challenges in materials science are directly linked to daily life, including the development of new energy storage systems that are alternatives to fossil fuels and the detoxification of toxic substances. However, these advances cannot be achieved in a day as scientific innovation is a process, which begins by understanding the phenomena that occur around us and building upon this knowledge.
Take dry cell batteries for example. To increase the capacity of manganese dry cells, alkaline dry cells, which are primary cells (single use), were developed. Over time, secondary cells (reusable by charging) became necessary, which led to the development of lithium-ion secondary cells. Today in the quest for the next generation of batteries, scientists strive to resolve current issues, including the development of shape-changeable dry cells using all solid batteries and polymers.
Behind every breakthrough are seeds to adequately meet society's needs. In materials research, one extraordinary seed is synchrotron radiation (or X-rays in the broad sense). This field investigates the structure of materials and their constituent elements to provide insight into the mechanisms of common everyday chemical reactions. Synchrotron radiation is a means to answer the questions such as: “What is this material?”, “How is this material formed?”, and “How can we examine this material?” Figure 1 shows several examples of the interactions between synchrotron radiation and materials.
The strength of synchrotron radiation is not that it creates new materials, but it is capable of probing the states of materials, and the gained knowledge can lead to innovations in materials science. Specifically, materials research uses synchrotron radiation experimental techniques in: 1) crystal structure analysis to elucidate the structures of materials, 2) X-ray absorption fine structure to clarify the chemical states and local structures of elements contained in materials, and 3) X-ray fluorescence analysis to examine the species and quantities of elements constituting materials. Although these techniques have been used with standard laboratory light sources for many years, combining them with synchrotron radiation has provided superior analyzing capabilities.
Synchrotron radiation at SPring-8 is highly brilliant, highly collimated, wavelength-tunable, and exhibits pulsing properties. These features lead to the following advantages, which cannot be realized in conventional laboratories. 1) Measurement time is significantly reduced (on the order of days to several minutes). 2) The appropriate wavelength (energy) can be selected. 3) Time-resolving measurements on the millisecond order are realized. 4) 2D analysis on the submicron order is possible.
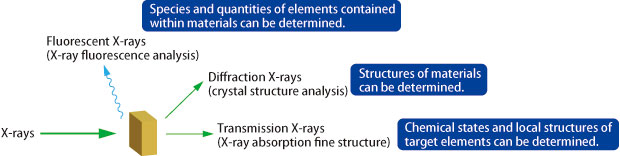
Fig. 1. Interactions between X-rays and materials.
Challenging the Limits of Nanobeam Technology
Focused nanobeams are expected to be a basic technology of synchrotron radiation, and should be indispensable in the study of nanoporous materials (Topic 24), battery materials (Topic 25), dioxin generation mechanisms (Topic 26), and decontamination mechanisms of heavy-metal contaminated soil (Topic 27). Previously most of these types of studies have been conducted using so-called “bulk” beams, but the obtained information is insufficient to provide details about the targets. Recent research has revealed that the reaction field, which determines the properties of materials, is in an extremely small region. Thus, to understand phenomena more accurately, the incorporation of nanobeam technology into these studies should be the key to success.
Thus, both synchrotron radiation and the development of light-focusing elements for X-rays have been advanced. Although there are several types of focusing elements, those best suited for the abovementioned experimental techniques in materials research have been developed based on total reflection mirrors and Fresnel diffraction. These elements themselves have been available since the 1950s, but applications to microbeams have only been available since the 1990s when second-generation synchrotron radiation facilities were realized. Second-generation synchrotron radiation sources such as those available at the Photon Factory at the High Energy Accelerator Research Organization in Tsukuba, Japan demonstrated that synchrotron radiation beams could be focused to 1 μm at 8.5 keV using a system that combines two total reflection mirrors, and could be further focused to 0.5 μm at 8.54 keV using diffraction-type mirrors called Fresnel zone plates. However, producing brilliant X-rays suitable for research requiring microbeams is difficult using second-generation synchrotron radiation facilities because the beam size (or spatial resolution) and brilliance of the focused X-rays conflict.
Fortunately, third-generation light sources such as those obtained at SPring-8 have resolved this issue. Third-generation light sources provide highly brilliant focused beams with a beam size on the nanometer order (nm = 10-9 m). Representative beam sizes are currently 30 nm at 15 keV using total reflection mirrors and 31 nm at 8 keV using Fresnel zone plates. Figure 2 summarizes the history behind the reduction of focused beam size. With the development of new light sources such as X-ray free electron laser (XFEL), it is expected that the limits of nanobeams will be challenged, which will consequently trigger new efforts in materials research.
In addition to attempts to develop focusing elements, some focusing technologies, which are in the high-energy X-ray regime, can only be pursued at SPring-8. For example, beam sizes of 0.4 μm at 80 keV have been realized using total reflection mirrors and 0.5 μm at 100 keV using Fresnel zone plates. Such microbeams utilized in the high-energy X-ray regime can effectively detect heavy metals (such as cadmium, uranium, antimony, and iodine), and due to recent attention, microbeams are receiving a growing interest from the viewpoint of materials research. For example, researchers have recently applied microbeams to analyze cadmium-accumulating plants using scanning microscopes as reported in Topic 27.
The new synchrotron light sources at SPring-8 have made extremely small beam sizes, which were a dream ten years ago, a reality. Based on the current pace of progress in research and technology, materials research using nanobeams should gain significant momentum in the not-so-distant future. For example, the chemical states within a few tens of nm of the surface layers of a single catalyst particle with a diameter of a few hundreds of nm and the distributions of metals in subcellular organelles (such as microsome and mitochondria) are both expected to be elucidated in the immediate future. Encouraged by the research projects introduced in this SPring-8 Scientific Achievements, materials research should advance into a new phase.
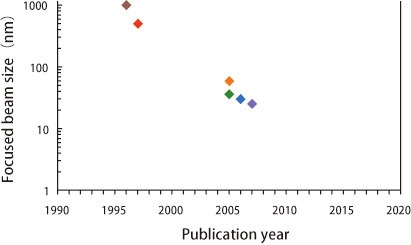
Fig. 2.History of focused beam size.